With Ultralight Lithium-Sulfur Batteries, Electric Airplanes Could Finally Take Off
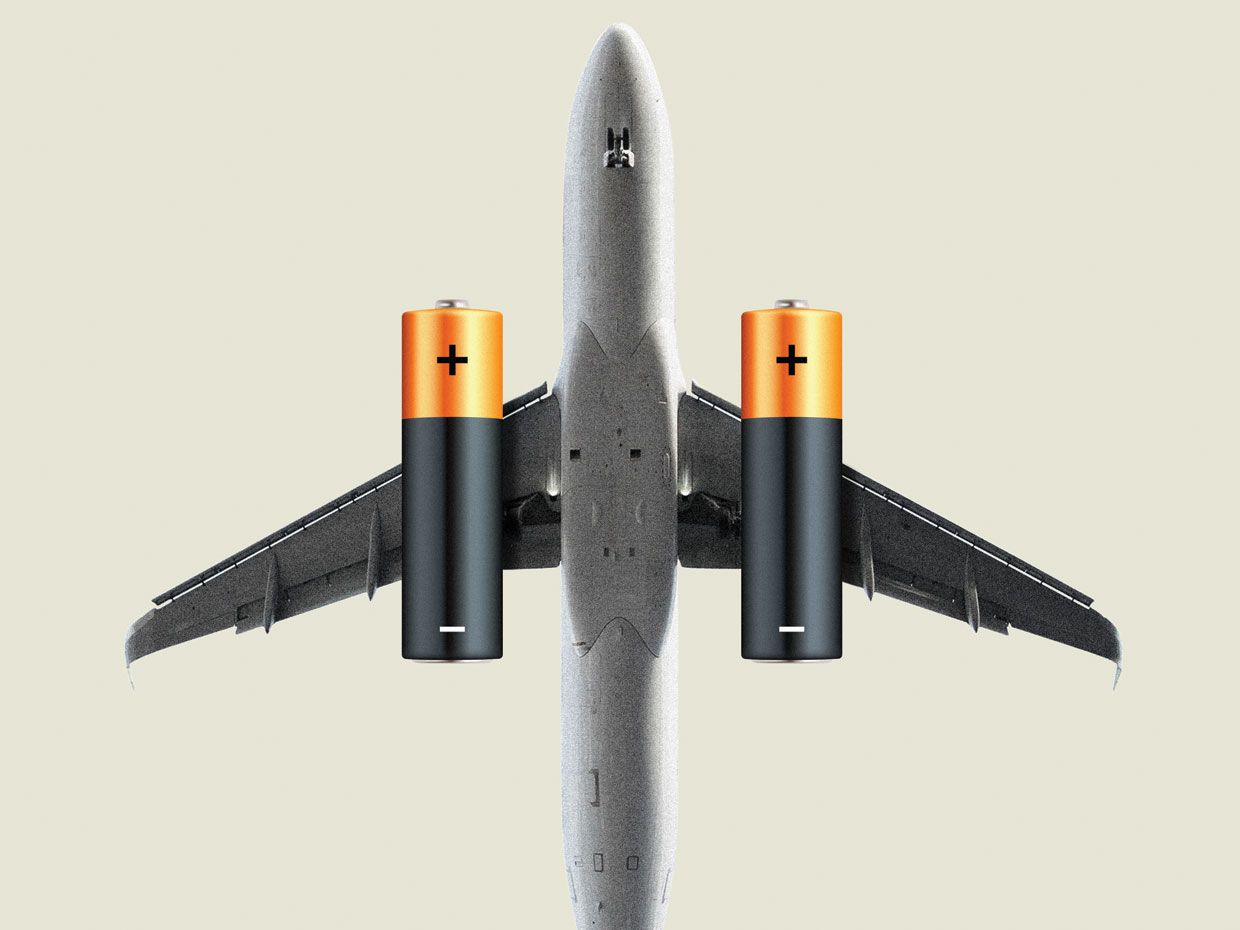
Electric aircraft are all the rage, with prototypes in development in every size from delivery drones to passenger aircraft. But the technology has yet to take off, and for one reason: lack of a suitable battery.
For a large passenger aircraft to take off, cruise, and land hundreds of kilometers away would take batteries that weigh thousands of kilograms-far too heavy for the plane to be able to get into the air in the first place. Even for relatively small aircraft, such as two-seat trainers, the sheer weight of batteries limits the plane's payload, curtails its range, and thus constrains where the aircraft can fly. Reducing battery weight would be an advantage not only for aviation, but for other electric vehicles, such as cars, trucks, buses, and boats, all of whose performance is also directly tied to the energy-to-weight ratio of their batteries.
For such applications, today's battery of choice is lithium ion. It reached maturity years ago, with each new incremental improvement smaller than the last. We need a new chemistry.
Since 2004 my company, Oxis Energy, in Oxfordshire, England, has been working on one of the leading contenders-lithium sulfur. Our battery technology is extremely lightweight: Our most recent models are achieving more than twice the energy density typical of lithium-ion batteries. Lithium sulfur is also capable of providing the required levels of power and durability needed for aviation, and, most important, it is safe enough. After all, a plane can't handle a sudden fire or some other calamity by simply pulling to the side of the road.
The new technology has been a long time coming, but the wait is now over. The first set of flight trials have already been completed.
Fundamentally, a lithium-sulfur cell is composed of four components:
- The positive electrode, known as the cathode, absorbs electrons during discharge. It is connected to an aluminum-foil current collector coated with a mixture of carbon and sulfur. Sulfur is the active material that takes part in the electrochemical reactions. But it is an electrical insulator, so carbon, a conductor, delivers electrons to where they are needed. There is also a small amount of binder added to ensure the carbon and sulfur hold together in the cathode.
- The negative electrode, or anode, releases electrons during discharge. It is connected to pure lithium foil. The lithium, too, acts as a current collector, but it is also an active material, taking part in the electrochemical reaction.
- A porous separator prevents the two electrodes from touching and causing a short circuit. The separator is bathed in an electrolyte containing lithium salts.
- An electrolyte facilitates the electrochemical reaction by allowing the movement of ions between the two electrodes.
These components are connected and packaged in foil as a pouch cell. The cells are in turn connected together-both in series and in parallel-and packaged in a 20 ampere-hour, 2.15-volt battery pack. For a large vehicle such as an airplane, scores of packs are connected to create a battery capable of providing tens or hundreds of amp-hours at several hundred volts.
Lithium-sulfur batteries are unusual because they go through multiple stages as they discharge, each time forming a different, distinct molecular species of lithium and sulfur. When a cell discharges, lithium ions in the electrolyte migrate to the cathode, where they combine with sulfur and electrons to form a polysulfide, Li2S8. At the anode, meanwhile, lithium molecules give up electrons to form positively charged lithium ions; these freed electrons then move through the external circuit-the load-which takes them back to the cathode. In the electrolyte, the newly produced Li2S8 immediately reacts with more lithium ions and more electrons to form a new polysulfide, Li2S6. The process continues, stepping through further polysulfides, Li2S4 and Li2S2, to eventually become Li2S. At each step more energy is given up and passed to the load until at last the cell is depleted of energy.
Recharging reverses the sequence: An applied current forces electrons to flow in the opposite direction, causing the sulfur electrode, or cathode, to give up electrons, converting Li2S to Li2S2. The polysulfide continues to add sulfur atoms step-by-step until Li2S8 is created in the cathode. And each time electrons are given up, lithium ions are produced that then diffuse through the electrolyte, combining with electrons at the lithium electrode to form lithium metal. When all the Li2S has been converted to Li2S8, the cell is fully charged.
This description is simplified. In reality, the reactions are more complex and numerous, taking place also in the electrolyte and at the anode. In fact, over many charge and discharge cycles, it is these side reactions that cause degradation in a lithium-sulfur cell. Minimizing these, through the selection of the appropriate materials and cell configuration, is the fundamental, underlying challenge that must be met to produce an efficient cell with a long lifetime.
Anatomy of a BatteryA lithium-sulfur cell goes through stages as it discharges [left]. In each stage, lithium ions in the electrolyte flow to the cathode, where they form polysulfides having ever higher sulfur-to-lithium ratios. Charging reverses the process. Cells are linked into battery packs, which themselves fit into a casing, along with battery-management devices.
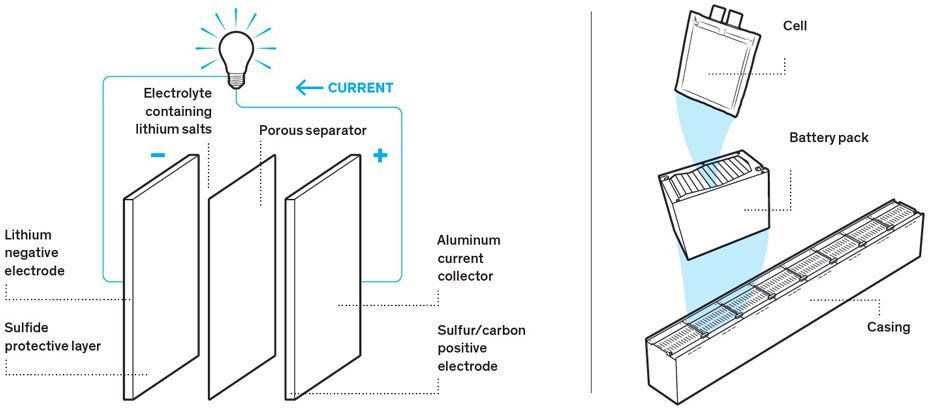
One great challenge for both lithium-ion and lithium-sulfur technologies has been the tendency for repeated charging and discharging cycles to degrade the anode. In the case of lithium ion, ions arriving at that electrode normally fit themselves into interstices in the metal, a process called intercalation. But sometimes ions plate the surface, forming a nucleus on which further plating can accumulate. Over many cycles a filament, or dendrite, may grow until it reaches the opposing electrode and short-circuits the cell, causing a surge of energy, in the form of heat that irreparably damages the cell. If one cell breaks down like this, it can trigger a neighboring cell to do the same, beginning a domino effect known as a thermal runaway reaction-in common parlance, a fire.
With lithium-sulfur cells, degradation of the lithium-metal anode is also a problem. However, this occurs via a very different mechanism, one that does not involve the formation of dendrites. In lithium-sulfur cells, uneven current densities on the anode surface cause lithium to be plated and stripped unevenly as the battery is charged and discharged. Over time, this uneven plating and stripping causes mosslike deposits on the anode that react with the sulfide and polysulfides in the electrolyte. These mosslike deposits become electrically disconnected from the bulk anode, leaving less of the anode surface available for chemical reaction. Eventually, as this degradation progresses, the anode fails to operate, preventing the cell from accepting charge.
Developing solutions to this degradation problem is crucial to producing a cell that can perform at a high level over many charge-discharge cycles. A promising strategy we've been pursuing at Oxis involves coating the lithium-metal anode with thin layers of ceramic materials to prevent degradation. Such ceramic materials need to have high ionic conductivity and be electrically insulating, as well as mechanically and chemically robust. The ceramic layers allow lithium ions to pass through unimpeded and be incorporated into the bulk lithium metal beneath.
We are doing this work on the protection layer for the anode in partnership with Pulsedeon and Leitat, and we're optimistic that it will dramatically increase the number of times a cell can be discharged and charged. And it's not our only partnership. We're also working with Arkema to improve the cathode in order to increase the power and energy density of the battery.
Indeed, the key advantage of lithium-ion batteries over their predecessors-and of lithium sulfur over lithium ion-is the great amount of energy the cells can pack into a small amount of mass. The lead-acid starter battery that cranks the internal combustion engine in a car can store about 50 watt-hours per kilogram. Typical lithium-ion designs can hold from 100 to 265 Wh/kg, depending on the other performance characteristics for which it has been optimized, such as peak power or long life. Oxis recently developed a prototype lithium-sulfur pouch cell that proved capable of 470 Wh/kg, and we expect to reach 500 Wh/kg within a year. And because the technology is still new and has room for improvement, it's not unreasonable to anticipate 600 Wh/kg by 2025.
When cell manufacturers quote energy-density figures, they usually specify the energy that's available when the cell is being discharged at constant, low power rates. In some applications such low rates are fine, but for the many envisioned electric aircraft that will take off vertically, the energy must be delivered at higher power rates. Such a high-power feature must be traded off for lower total energy-storage capacity.
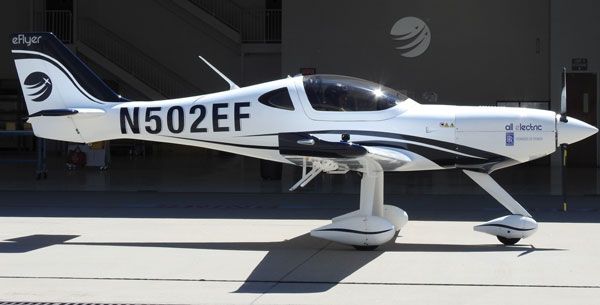
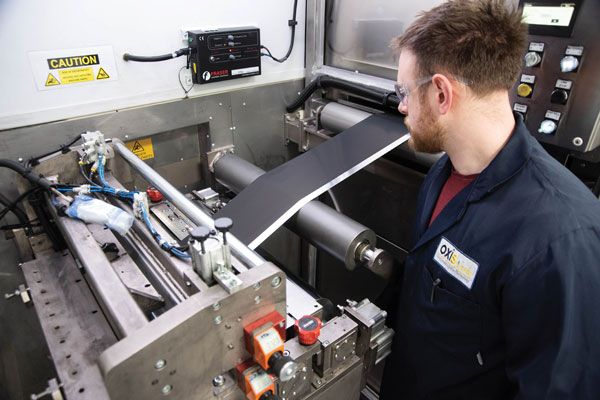
Furthermore, the level of energy density achievable in a single cell might be considerably greater than what's possible in a battery consisting of many such cells. The energy density doesn't translate directly from the cell to the battery because cells require packaging-the case, the battery management system, and the connections, and perhaps cooling systems. The weight must be kept in check, and for this reason our company is using advanced composite materials to develop light, strong, flameproof enclosures.
If the packaging is done right, the energy density of the battery can be held to 80 percent of that of the cells: A cell rated at 450 Wh/kg can be packaged at more than 360 Wh/kg in the final battery. We expect to do better by integrating the battery into the aircraft, for instance, by making the wing space do double duty as the battery housing. We expect that doing so will get the figure up to 90 percent.
To optimize battery performance without compromising safety we rely, first and foremost, on a battery management system (BMS), which is a combination of software and hardware that controls and protects the battery. It also includes algorithms for measuring the energy remaining in a battery and others for minimizing the energy wasted during charging.
Like lithium-ion cells, lithium-sulfur cells vary slightly from one another. These differences, as well as differences in the cells' position in the battery pack, may cause some cells to consistently run hotter than others. Over time, those high temperatures slowly degrade performance, so it is important to minimize the power differences from cell to cell. This is usually achieved using a simple balancing solution, in which several resistors are connected in parallel with a cell, all controlled by software in the BMS.
Even when charging and discharging rates are kept within safe limits, any battery may still generate excessive heat. So, typically, a dedicated thermal-management system is necessary. An electric car can use liquid cooling, but in aviation, air cooling is much preferred because it adds less weight. Of course, the battery can be placed at a point where air is naturally moving across the surface of the airplane-perhaps the wing. If necessary, air can be shunted to the battery through ducts. At Oxis, we're using computational modeling to optimize such cooling. For instance, when we introduced this technique in a project for a small fixed-wing aircraft, it allowed us to design an effective thermal-management system, without which the battery would reach its temperature limits before it was fully discharged.
As noted above, a battery pack is typically arranged with the cells both in parallel and in series. However, there's more to the arrangement of cells. Of course, the battery is a mission-critical component of an e-plane, so you'll want redundancy, for enhanced safety. You could, for instance, design the battery in two equal parts, so that if one half fails it can be disconnected, leaving the aircraft with at least enough energy to manage a controlled descent and landing.
Another software component within the BMS is the state-of-charge algorithm. Imagine having to drive a car whose fuel gauge had a measurement error equivalent to 25 percent of the tank's capacity. You'd never let the indicator drop to 25 percent, just to make sure that the car wouldn't sputter to a halt. Your practical range would be only three-quarters of the car's actual range. To avoid such waste, Oxis has put a great emphasis on the development of state-of-charge algorithms.
In a lithium-ion battery you can estimate the charge by simply measuring the voltage, which falls as the energy level does. But it's not so simple for a lithium-sulfur battery. Recall that in the lithium-sulfur battery, different polysulfides figure in the electrochemical process at different times during charge and discharge. The upshot is that voltage is not a good proxy for the state of charge and, to make things even more complicated, the voltage curve is asymmetrical for charge and for discharge. So the algorithms needed to keep track of the state of charge are much more sophisticated. We developed ours with Cranfield University, in England, using statistical techniques, among them the Kalman filter, as well as neural networks. We can estimate state of charge to an accuracy of a few percent, and we are working to do better still.
All these design choices involve trade-offs, which are different for different airplanes. We vary how we manage these trade-offs in order to tailor our battery designs for three distinct types of aircraft.
- High-altitude pseudo satellites (HAPS) are aircraft that fly at around 15,000 to 20,000 meters. The hope is to be able to fly for months at a time; the current record is 26 days, set in 2018 by the Airbus Zephyr S. By day, these aircraft use solar panels to power the motors and charge the batteries; by night, they fly on battery power. Because the 24-hour charge-and-discharge period demands only a little power, you can design a light battery and thus allow for a large payload. The lightness also makes it easier for such an aircraft to fly far from the equator, where the night lasts longer.
- Electric vertical take-off and landing (eVTOL) aircraft are being developed as flying taxis. Lilium, in Germany, and Uber Elevate, among others, already have such projects under way. Again, weight is critical, but here the batteries need not only be light but must also be powerful. Oxis has therefore developed two versions of its cell chemistry. The high-energy version is optimized in many aspects of the cell design to minimize weight, but it is limited to relatively low power; it is best suited to HAPS applications. The high-power version weighs more, although still significantly less than a lithium-ion battery of comparable performance; it is well suited for such applications as eVTOL.
- Light fixed-wing aircraft: The increasing demand for pilots is coming up against the high cost of training them; an all-electric trainer aircraft would dramatically reduce the operation costs. A key factor is longer flight duration, which is enabled by the lighter battery. Bye Aerospace, in Colorado, is one company leading the way in such aircraft. Furthermore, other companies-such as EasyJet, partnered with Wright Electric-are planning all-electric commercial passenger jets for short-haul, 2-hour flights.
Three factors will determine whether lithium-sulfur batteries ultimately succeed or fail. First is the successful integration of the batteries into multiple aircraft types, to prove the principle. Second is the continued refinement of the cell chemistry. Third is the continued reduction in the unit cost. A plus here is that sulfur is about as cheap as materials get, so there's reason to hope that with volume manufacturing, the unit cost will fall below that of the lithium-ion design, as would be required for commercial success.
Oxis has already produced tens of thousands of cells, and it is currently scaling up two new projects. Right now, it is establishing a manufacturing plant for the production of both the electrolyte and the cathode active material in Port Talbot, Wales. Later, the actual mass production of lithium-sulfur cells will begin on a site that belongs to Mercedes-Benz Brazil, in Minas Gerais, Brazil.
This state-of-the-art plant should be commissioned and operating by 2023. If the economies of scale prove out, and if the demand for electric aircraft rises as we expect, then lithium-sulfur batteries could begin to supplant lithium-ion batteries in this field. And what works in the air ought to work on the ground, as well.
This article appears in the August 2020 print issue as Ultralight Batteries for Electric Airplanes."
About the AuthorMark Crittenden is head of battery development and integration at Oxis Energy, in Oxfordshire, U.K.